Particle Physics: Navigating a Bright Future Amid Cosmic Mysteries
Written on
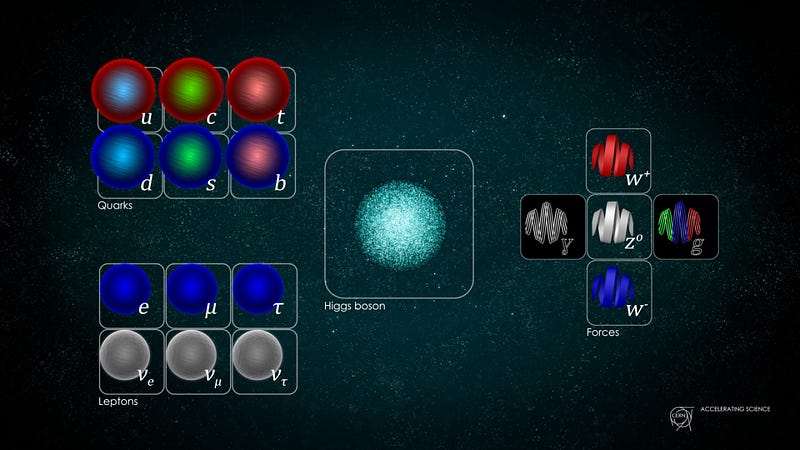
Particle physics has recently begun to emerge from a tumultuous period. Ten years back, the field seemed chaotic. The Large Hadron Collider (LHC) had started operation and confirmed the existence of the Higgs boson, the last particle anticipated by the Standard Model. However, it did not provide insights into any other theories aiming to extend beyond this model. Fermilab, once a frontrunner in high-energy physics, permanently closed its main accelerator. This left many significant questions, including:
- The cause of neutrino mass,
- The essence of dark matter,
- The source of matter-antimatter imbalance,
These questions appeared to be at a standstill, with little advancement in experimental or theoretical realms.
Furthermore, particle physicists were embroiled in disagreements over their future direction. Would investing in a new, more powerful accelerator than the LHC yield sufficient returns? How could neutrino behavior be examined to better understand their mass? Were they fully exploiting the link between particle physics and cosmology? Were their dark matter investigations based on misguided theories? And were they doing enough to support early-career researchers and smaller experimental projects?
Finally, the Particle Physics Projects Prioritization Panel (P5) report has provided a unified strategy for the next decade, addressing these concerns while fostering a nurturing environment for particle physics in the United States and globally. Here’s a summary of the key points.
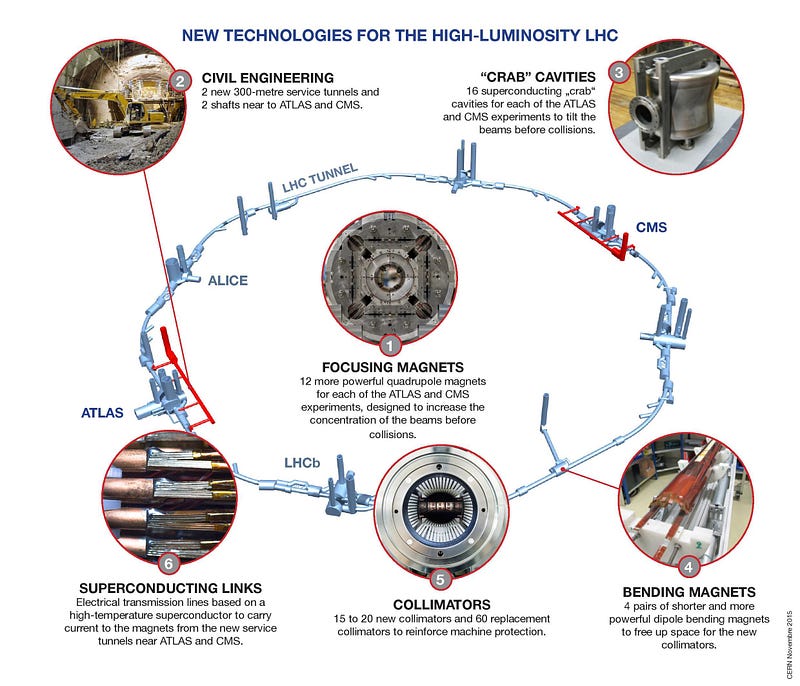
A Misguided Perspective on Particle Physics
A prevalent, yet simplistic, perspective on particle physics persists among many. It claims that:
- The Standard Model has been established since the 1960s, resulting in stagnation in the field.
- Fundamental science has reached its limit, with theorists pursuing flawed ideas.
- Experimentalists continue to seek evidence for these flawed ideas, only to find increasingly precise “null” results.
- Overall, meaningful progress in particle physics has ceased.
This viewpoint fails to grasp the essence of particle physics and the nature of scientific research.
The Standard Model is not a definitive theory like Newtonian gravity or Einstein’s theory of relativity. Rather, it serves as a structural framework that describes three generations of fermions, categorized into quarks and leptons, alongside bosons that mediate the strong, weak, and electromagnetic forces. To fully understand how the Standard Model operates in our universe, we must conduct measurements to determine the masses of fundamental particles, properties of quark mixing, and the types of symmetry violations present.
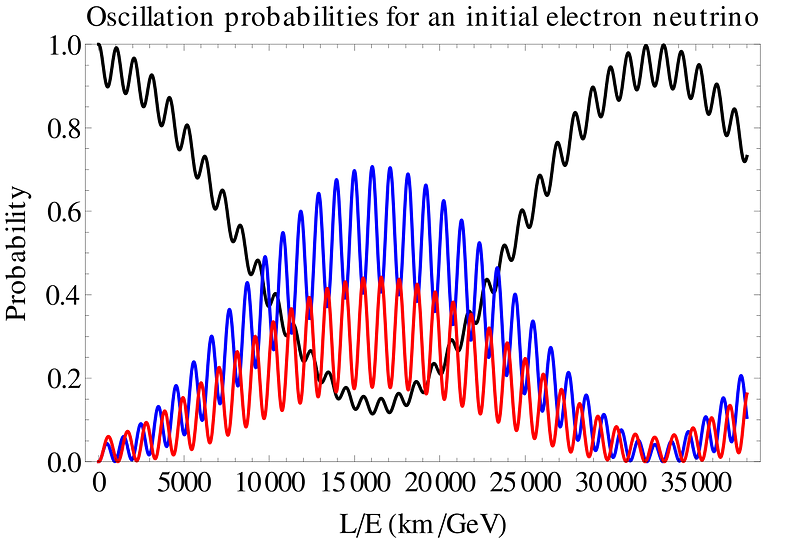
Understanding the Standard Model and Beyond
It's also clear that the Standard Model does not encompass the entirety of reality. For example, we know that:
- The universe was once significantly more energetic than the highest-energy collisions generated by the LHC.
- Dark matter and dark energy pervade the universe, yet neither is explained by the Standard Model.
- The universe is predominantly composed of matter, despite a symmetric understanding of particle interactions that suggests otherwise.
- Various properties of our universe, such as neutrino masses and the low rest masses of fundamental particles, remain unexplained.
Extensive investigations into plausible scenarios have been made to address these mysteries, with experimental particle physics placing critical constraints on theories that extend beyond the Standard Model. The original versions of supersymmetry (SUSY) and Peccei-Quinn symmetry, as well as the Grand Unified Theory of SU(5), have been ruled out as solutions.
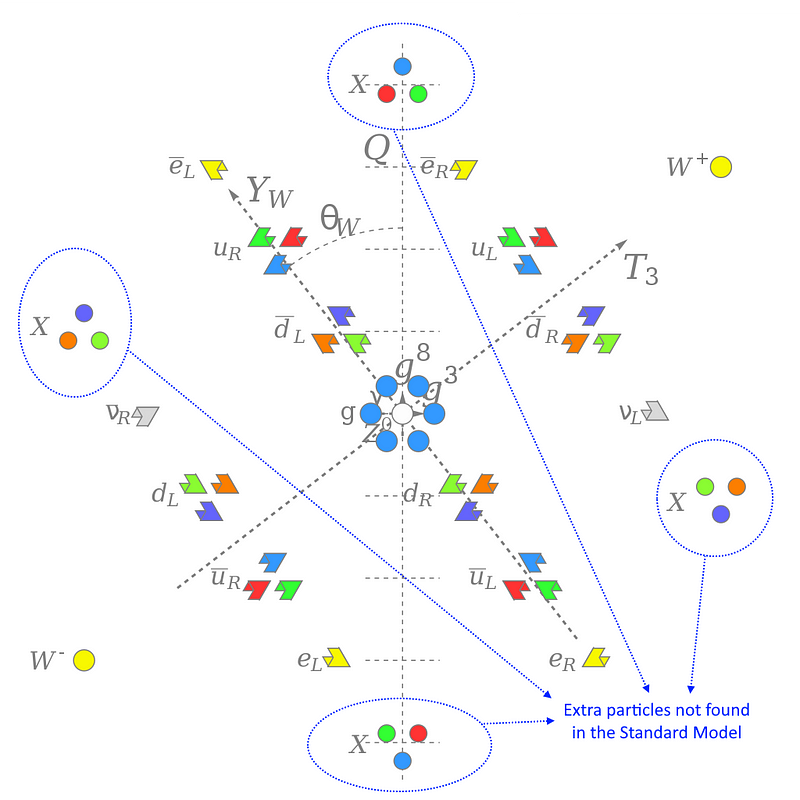
Tackling Major Particle Mysteries
Despite the persistence of these enigmas, they remain crucial to both particle physics and cosmology. The most extreme conditions in cosmic history occurred in the initial moments following the Big Bang. The earliest evidence of the universe's quantum nature arose during cosmic inflation, a phase that set the stage for the hot Big Bang. Many of the puzzles we contemplate today, such as:
- Dark matter,
- Dark energy,
- The nature of inflation,
- The origin of neutrino mass,
- The properties of the Higgs boson,
Likely have their resolutions rooted in these early cosmic events.
To uncover solutions, a multifaceted approach is essential. High-energy colliders will play a role, but so will refined methods such as searching for rare interactions, measuring neutrino oscillations, cosmic observations sensitive to ancient imprints, and developing innovative technologies and laboratory experiments. To truly understand the workings of nature, we must ask the right questions.
The P5 recommendations successfully encapsulate this ethos.
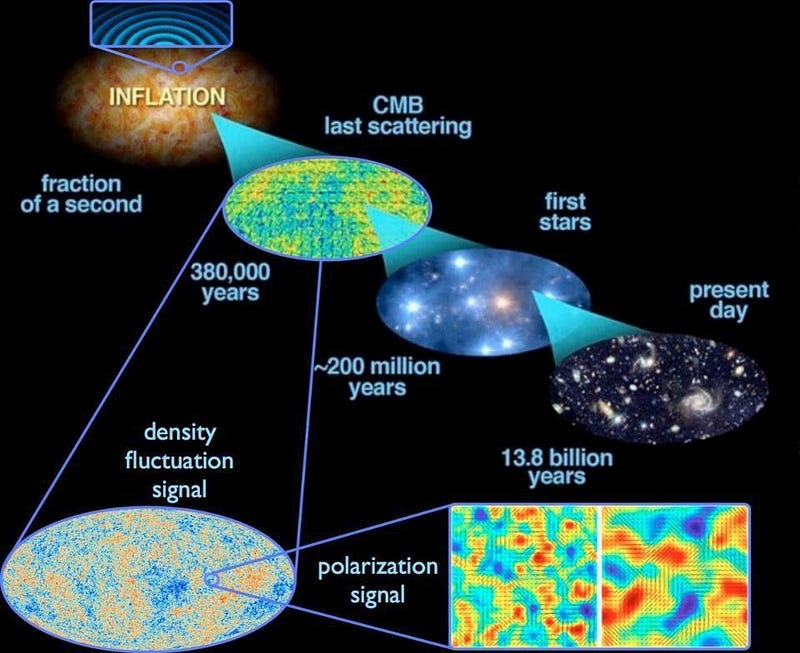
High-Impact Investments with a Rational Budget
The overarching goals of a scientific discipline like particle physics are fourfold:
- To facilitate research addressing significant contemporary questions, asking nature pivotal inquiries that may enhance our understanding.
- To strike a balance between theoretical pursuits and experimental measurements.
- To establish a roadmap for future research and support upcoming researchers.
- To cater to various aspects of the community, from large collaborations to smaller projects and technological innovations.
While the P5 report aptly balances these competing priorities, the most exciting aspects for many lie in the major scientific initiatives prioritized.
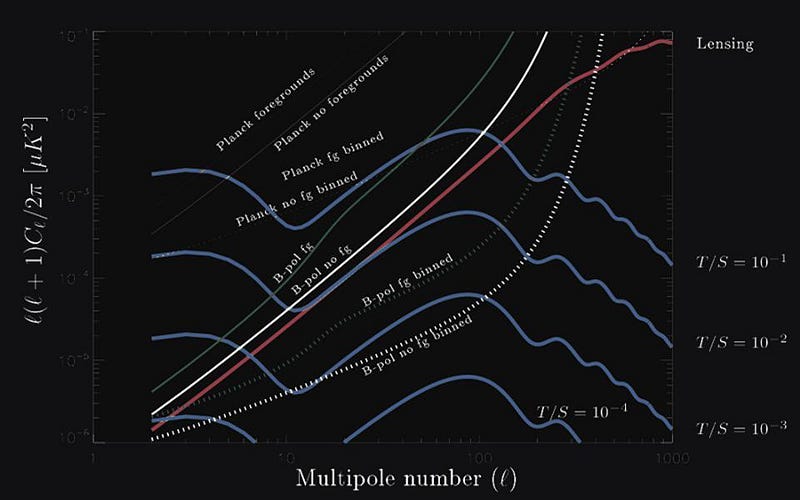
At the intersection of particle physics and cosmology lies the quantum imprints left on cosmic scales by the final moments of inflation. While we’ve made strides in understanding the density fluctuations caused by inflation, we must also investigate gravitational wave fluctuations, known as tensor modes.
Next-generation efforts to measure these gravitational wave signals, termed CMB-S4 experiments, are among the top priorities recommended by the P5 report.
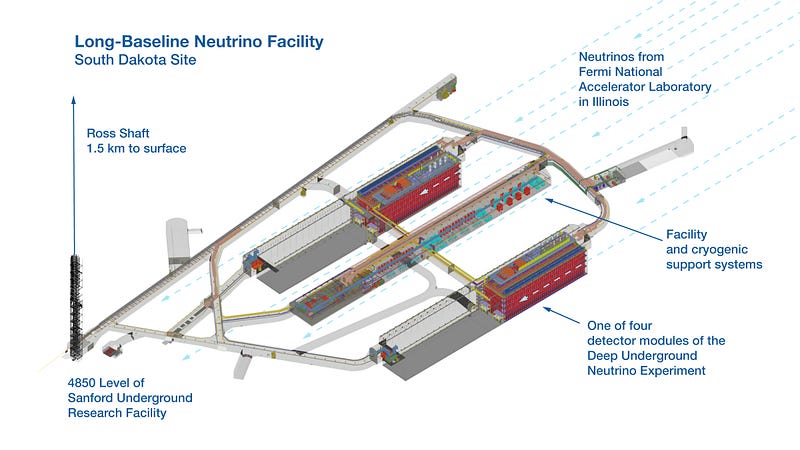
One of the most significant projects underway in the United States is DUNE: the Deep Underground Neutrino Experiment. DUNE aims to:
- Generate a particle beam of unstable pions,
- Allow these pions to decay into muons and muon neutrinos,
- Send these neutrinos through the Earth for interaction with its matter,
- Measure the neutrinos using a specialized detector known as a time-projection chamber.
This long-baseline neutrino experiment represents a significant advancement over current efforts and is essential for probing the nature of neutrinos, which oscillate between different types as they travel.
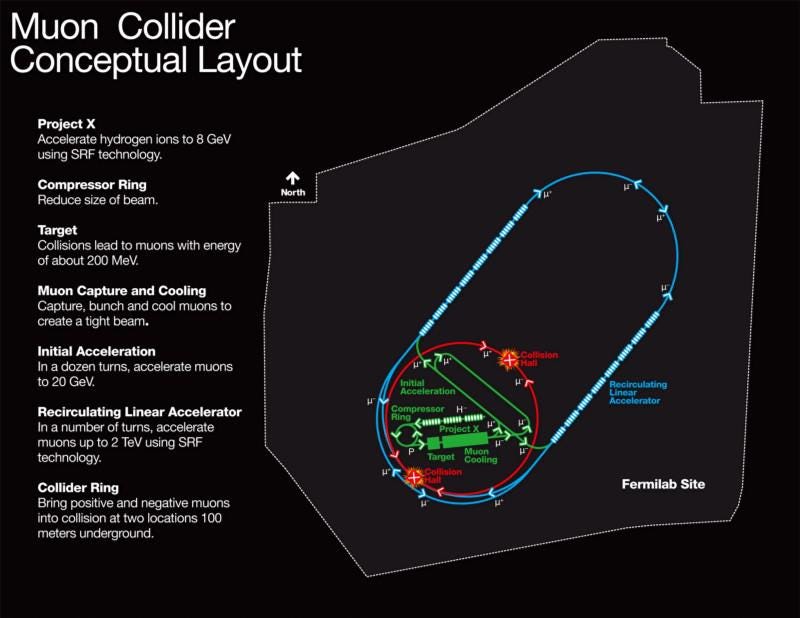
The P5 report wisely recommends a cautious approach to investing in proposed next-generation colliders. While it acknowledges the need for a machine to study the Higgs boson, it suggests supporting the technological development of various accelerator designs to determine which holds the most promise.
Three main accelerator designs are currently under consideration:
- A future circular collider with a larger ring than the LHC, capable of colliding electrons or hadrons for high-energy collisions.
- A linear collider for pristine electron-positron collisions, though it may not push energy boundaries.
- A circular muon collider for high-energy collisions, albeit with fewer total interactions due to muon instability.
Advancements in energy gain or muon luminosity could favor one design over others.
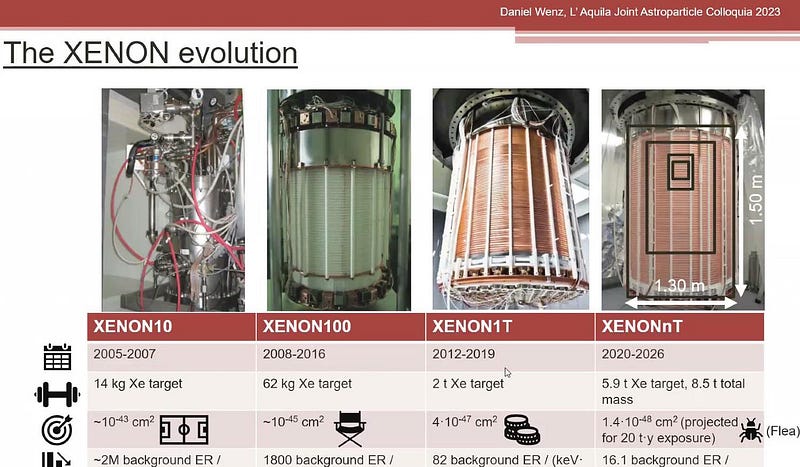
Equally prioritized is the establishment of at least one next-generation dark matter direct detection experiment. Contrary to common belief, dark matter searches have not been failures; rather, they guide scientists on where to look. Over the past three decades, sensitivity to exotic particles has dramatically increased, underscoring the effectiveness of these searches.
The aim of the next-generation experiments is to become sensitive enough to detect the “neutrino fog,” which consists of cosmic neutrinos, those from terrestrial radioactivity, and solar neutrinos.
Particle physicists are broadening their scope, learning from past assumptions regarding dark matter to encompass a wider array of possibilities.
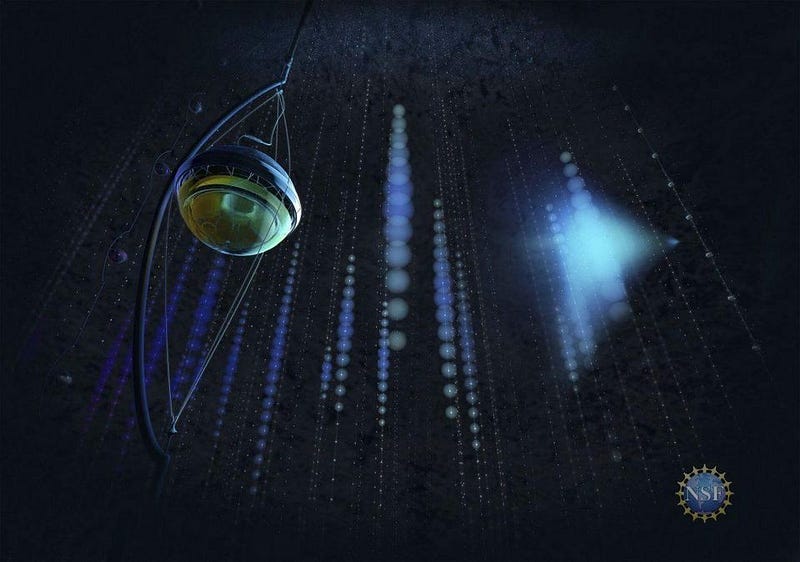
Additionally, multi-messenger astronomy is gaining recognition in the particle physics community. Currently, three distinct signal types are detectable from cosmic sources:
- Light in various forms, from gamma rays to radio waves,
- Gravitational waves, detected by LIGO, Virgo, and pulsar timing,
- Cosmic particles, including neutrinos from solar, galactic, and extragalactic sources.
While the first two signal types largely pertain to astronomy, cosmic particle detection is of paramount interest to particle physicists.
At the South Pole, the IceCube detector excels at detecting cosmic neutrinos. It has produced a galactic map in neutrinos and detected neutrinos from an extragalactic source. Enhancing IceCube’s capabilities will enable groundbreaking studies of neutrino properties and facilitate a comprehensive multi-messenger astronomy program. If a supernova occurs within the Local Group, the next-generation IceCube will yield remarkable scientific insights.
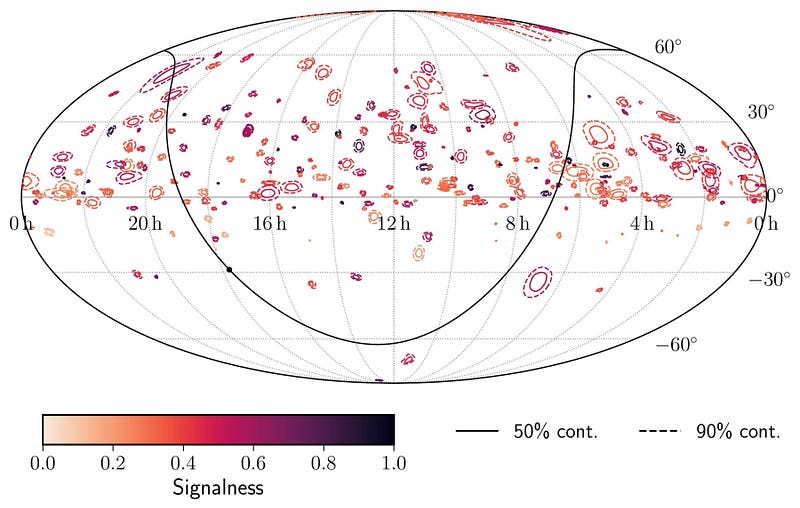
Conclusion & Summary
This strategic approach emphasizes a balanced investment within a reasonable budget. Particle physicists are not advocating for a drastic funding increase but are maintaining an annual budget from the US Department of Energy between $1 billion and $2 billion over the next decade. They aim to support early-career scientists and diverse small experiments across national laboratories and universities. Rather than presenting an exhaustive wish-list or demanding a single flagship project, they are focused on targeted investments that maximize scientific returns.
Investing in fundamental research is crucial for any society's scientific future. Such investments foster economic activity, technological advancements, and global scientific leadership, yielding substantial long-term returns. The P5 report illustrates that particle physicists are learning from past mistakes and refraining from making overambitious promises about future discoveries. With the pathway outlined in the P5 report, particle physics has the potential for a vibrant future in the United States.